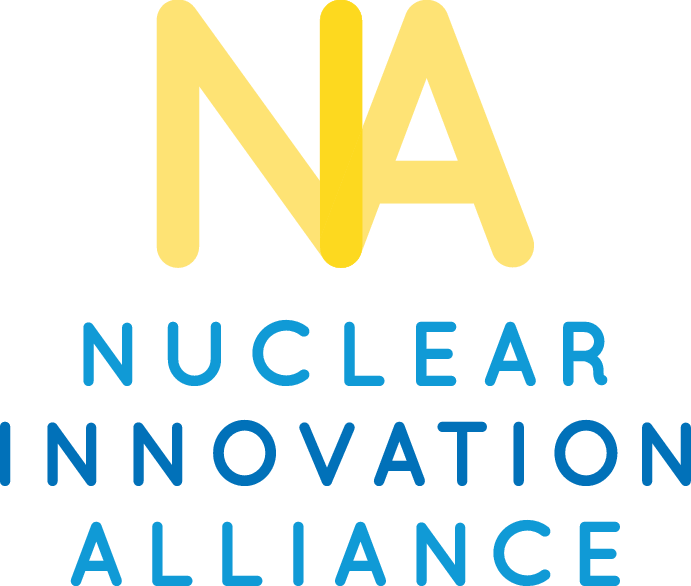
WASHINGTON, D.C. Nuclear energy is the largest source of carbon-free power in the United States and second largest source globally. As countries around the world consider nuclear energy for their 21st century decarbonization needs, one important question is whether there is enough uranium fuel for nuclear energy to play a greatly expanded role in global energy supply. While uranium is not a completely unlimited resource, currently known uranium resources and reserves are sufficient to power decarbonized global energy systems in the 21st century and beyond.
Origin of Uranium and Its Use as a Fuel
As the heaviest element found in nature, uranium’s cosmogenic origin is in supernova explosions that occurred long ago. These supernovas created many heavy elements, including thorium and uranium, which were spread across the dust cloud that would become our solar system. The formation of Earth and sustained geological processes led to the concentration of uranium into accessible terrestrial resources. Today’s uranium mines have concentrations of uranium (ore grades) ranging from less than 0.1% to as much as 20%.
Supplying fuel for a nuclear reactor that can produce carbon-free energy first requires the mining and processing of natural uranium. Naturally occurring uranium is primarily a mix of two different uranium isotopes: uranium-238 (99.3%) and uranium-235 (0.7%). Uranium-235 is considered a fissile[1] nuclide because it can absorb a neutron of any energy and be used to sustain a fission chain reaction. Conversely, uranium-238 can only support a fission chain reaction under specific conditions and is considered a fertile[2] nuclide. Therefore, to be used for nuclear energy, most reactors require uranium fuel with a higher concentration (percentage) of uranium-235 than naturally occurring uranium to sustain a continued fission chain reaction. The process of achieving this higher concentration of uranium-235 in fuel is called enrichment. For example, modern light water reactors require uranium enriched to approximately 3-5% uranium-235.
In modern light water reactors, the initial fuel loading essentially consists of only uranium-235 and uranium-238 but as the reactor is operated, the fuel composition begins to change. When a nuclear reactor is operating, neutrons released by fissioning atoms can be absorbed by uranium-238 atoms and, after a series of radioactive decays, transmute (transform) into a different isotope, plutonium-239 (see Figure 1). Plutonium-239 is not found naturally on earth but is a fissile isotope capable of sustaining fission chain reactions in nuclear reactors. This new plutonium-239 atom can then fission in the reactor fuel and be used to sustain continued chain reactions in the reactor core. Thus, while initial uranium-235 fuel enrichment is needed for light water reactors, transmutation and subsequent fission of uranium-238 indirectly serves as a fuel supply. Fission of plutonium-239 atoms accounts for about one third of total reactor energy output throughout the lifetime of a fuel assembly.[3]
Figure 1. Conversion of fertile uranium-238 to fissile plutonium-239
Other types of reactors, such as liquid metal or sodium cooled reactors, can more effectively fission uranium-238 atoms as well as transmute greater amounts of uranium-238, leading to a greater relative consumption between the two isotopes. However, in these reactors, a higher concentration of fissile material (e.g., uranium-235, plutonium-239) is typically needed to start the chain reaction and begin the uranium-to-plutonium transmutation process. Characteristics of these and other reactor designs are covered in the Nuclear Innovation Alliance’s publication, Advanced Nuclear Reactor Technology: A Primer.
The unit tU, meaning tons of raw uranium (99.3% uranium-238 and 0.7% uranium-235), is commonly used to characterize the uranium fuel supply.
Uranium Supply and Demand
The authoritative global source on uranium supply is the “Redbook,” a joint publication of the International Atomic Energy Agency (IAEA) and the OECD Nuclear Energy Agency (NEA). The most recent version of their analysis found that as of 2019, global nuclear power plants require 59,200 tU annually. In looking at current high side cases, IAEA and NEA estimated that global nuclear power production could increase more than 50% by 2040, requiring 100,200 tU each year. Comparably, an assessment by the World Nuclear Association (WNA) found in a high scenario the world could need as much as 156,500 tU by 2040. It is conceivable that with very high growth in nuclear power generation to fight climate change, annual global demand could exceed 200,000 or even 250,000 tU by the latter half of the 21st century.
Uranium mines around the world produce between 50,000 and 60,000 tU annually. Figure 2 shows historic uranium demand and production worldwide. Utilization of uranium stockpiles in the 1990s significantly reduced the need for worldwide uranium production despite increasing demand. Continued use of historic stockpiles of civilian uranium, as well as material recovered from the decommissioning of nuclear weapons, have contributed to a fluctuating uranium market.
Figure 2. Worldwide uranium requirements and production (source: IAEA/NEA)
Uranium supply and demand are mediated by prices. Due to the persistent oversupply and stockpiles of uranium, uranium prices have generally been low for the last several decades. This has disincentivized the development of new mines, as well as reduced utilization of existing mines. Since 2008, IAEA and NEA found that global production “varied between 70 and 90% of full production capability.” At full production capability, existing, committed, planned, and prospective capacity could produce as much as 80,000 tU annually in the 2020s. In high demand scenarios, higher prices may be needed to support the development of future mines to match growth in nuclear energy. Accordingly, the ultimate question in terms of long-term fuel security is whether there are sufficient resources for future nuclear energy demand and at what prices. What resources and reserves are available globally?
Global Resources and Reserves of Uranium
For a long time, there were concerns about the theory of “peak oil” — the idea that we would hit maximum production of oil and then decline because of exhausted reserves. Over time, however, technology improvements have led to more resources becoming extractable at economically viable rates, delaying (or perhaps preventing) society from hitting “peak oil”. Similarly, although there may be concerns about “peak uranium” or uranium resource depletion, a review of identified, economically recoverable reserves indicates that there is sufficient uranium fuel supply for any conceivable 21st century clean energy need.[4]
Resources are generally categorized based on whether they are measured or inferred and the price levels at which they are economic to produce. As of 2019, IAEA and NEA found that total measured resources available to mine at prices less than $130/kgU (the highest price of the last twenty years) are 3.8 million tU. They also found additional inferred resources of 2.4 million tU, making a total level of 6.2 million tons of uranium likely available for future markets at moderate price levels. This is enough to supply 100 years of current demand, and more than 40 years in high demand scenarios. An additional 1.8 million tU is available at prices between $130/kgU and $260/kgU.
This estimate of uranium availability, however, only reflects the known reserves. Decades of persistently low uranium prices have limited commercial prospecting and exploration for uranium deposits worldwide. Additionally, there has been limited investment in mining technology that could make additional uranium deposits economically viable. Over time, reserves of a given resource can grow due to improved prospecting and technology, even if there is significant demand for the resource. For example, when IAEA and NEA conducted their analysis, they found that identified, economically recoverable uranium reserves globally actually increased between 2017 and 2019, despite two years of increasing nuclear energy production worldwide and a lack of substantial investment in uranium deposit exploration[5].
Beyond uranium deposits currently accessible at commercially viable prices and those that could be accessed with additional prospecting and technology, the potential future supply of uranium is vast. There are many low-grade uranium ore deposits that are not currently considered commercially viable that, in total, contain many times the total uranium inventory of currently evaluated higher ore-grade uranium resources. At the most extreme, there is as much as 4 billion tU in the world’s oceans, which could be accessible with future technologies and appropriate investment. At a maximum theoretical level, this could supply thousands or tens of thousands of years of supply for nuclear energy.
Uranium Prices and Reactor Economics
Although higher prices for raw uranium will eventually end up increasing the cost of nuclear energy, the overall effects are likely to be limited. Nuclear energy is a capital-intensive technology where fuel costs play only a small role in the overall delivered cost of energy. Further, fuel costs for reactors consist of more than just fuel; they also consist of fuel enrichment, fabrication, transportation, and other costs. An analysis by the WNA found that increasing uranium ore costs from $30/kgU (the average price for the last twenty years) to $130/kgU (the maximum price of the last twenty years) would increase fuel costs for a nuclear plant from $5/MWh to $10/MWh. A fuel cost increase of $5/MWh can be compared with the average existing U.S. nuclear power plant annual operating and fuel costs of around $30/MWh. These higher fuel prices could incentivize further innovation to reduce fuel costs.
Alternative Sources of Fuel Supply for Nuclear Energy
Most of this discussion has focused on fuel supply for large, conventional light-water reactors. Much of the expected growth in nuclear energy required to support high decarbonization scenarios are likely to be advanced nuclear reactors. While the fuel supply of light-water small modular reactors is likely to resemble that of conventional reactors, other advanced reactors feature innovations in design and fuel cycles that could greatly reduce pressures on uranium supply. Certain sodium, molten salt, liquid metal, or gas reactors (fast-spectrum reactors) can more effectively utilize uranium resources than light-water reactors by producing and consuming higher levels of plutonium-239 from otherwise unconsumed uranium-238.
Additional recycling or reprocessing of spent nuclear fuel from existing reactors could also be used to reduce future pressures on uranium supply. Used fuel from fission fuel cycles still contain significant fractions of fissile and fertile material. Build-up of neutron-absorbing fission products in used fuel during operation makes continued use of the spent fuel infeasible in existing LWRs. New reactor technologies, however, can be designed to make use of the remaining fissile and fertile isotopes in the fuel (direct recycling), or the fuel can be chemically separated (indirect reprocessing) to remove the fission products and make uranium and plutonium isotopes available for reuse for new reactor fuel. Fuel recycling or reprocessing could enable production of up to 30% more energy from the original uranium in future nuclear energy production without mining any additional uranium.
If the world greatly expands nuclear energy for decarbonization purposes and begins to reach uranium resource limitations in the 2200s or later, the world could also turn to thorium-fueled fission reactors. The main isotope of thorium, thorium-232, is fertile. Like uranium-238. thorium-232 fuel can absorb neutrons and after a series of radioactive decays, transmute into the fissile uranium-233. This enables a nuclear fuel cycle, independent of uranium mining, where thorium-232 can be transformed into fissile nuclear fuel during operation in specific advanced reactor designs.
Thorium terrestrial abundance is several times greater than raw uranium. There have not yet been economic pressures to pursue thorium fuel cycles. As a result, thorium resources globally remain largely undiscovered. This potential additional fuel availability could further extend nuclear fuel availability well into the next millennium. While the use of thorium as a fission fuel has additional design and operational challenges, the natural abundance of terrestrial thorium largely eliminates fuel availability as a constraint on reactor development and deployment.
[1] Fissile: A nuclide that can undergo fission after capturing a neutron of any energy (e.g., uranium-235, plutonium-239).
[2] Fertile: Material that can absorb a neutron, radioactively transmute or convert into fissile material, and then undergo fission (e.g., uranium-238, thorium-232).
[3] http://www.world-nuclear.org/information-library/nuclear-fuel-cycle/fuel-recycling/plutonium.aspx